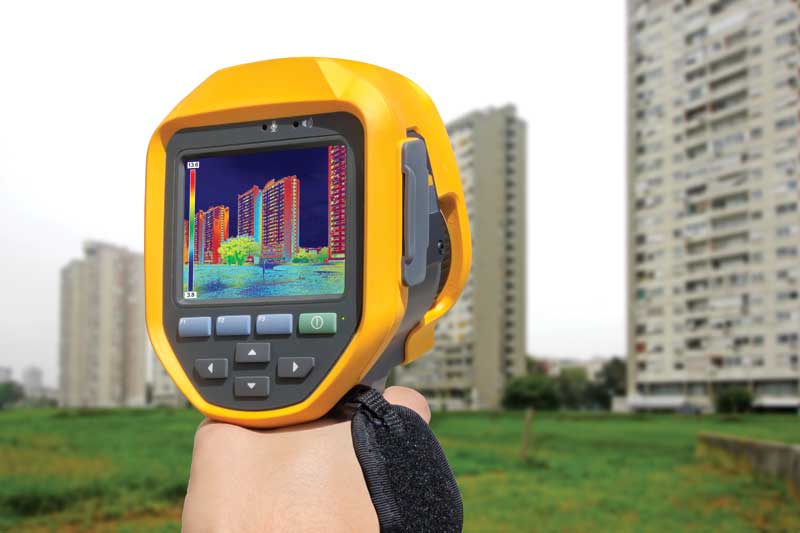
by Sarah K. Flock, CDT, AIA and Carole Ceja, NCARB, RRC
Many designers and specifiers understand controlling air, vapor, and thermal transfer helps mitigate moisture problems within the building envelope. Moisture accumulation is a performance adversary that can lead to structural deterioration, finish damage, organic growth, and reduced building longevity (Figure 1). However, navigating fundamentals, code requirements, and industry trends related to these transfer mechanisms can be complex.
The 2015 International Codes (I-Codes) were recently released; as of this writing, six states have adopted the 2015 International Energy Conservation Code (IECC) and International Building Code (IBC), with many more expected to join in the months to come. Even when designs meeting current codes are achieved, moisture issues can result. This article explores the impact of recent code changes, highlights various provisions’ potential limitations, and presents examples of ‘gaps’ between codes and real-world performance as they relate to the topics of air, vapor, and thermal control.
Thermal transfer
To incorporate the concept of thermal control in design, the fundamentals of heat transfer must be understood. Heat can transfer in various ways, such as conduction, convection, or radiation; it moves from areas of high to low temperatures independent of orientation.
Conduction is the most familiar mode and is the flow of heat through solid materials, such as window frames or metal studs. The amount of heat transfer via conduction depends on the material’s conductivity, mass, and configuration.
Convection is the transfer of heat through a gas or liquid, such as air, and can occur either naturally or by force. Natural convection occurs in the wake of differing densities, exemplified in the old adage of hot air rising. Forced convection is based on similar principles, but may deal with increased rates of air movement generated by forces from outside (e.g. wind) or inside (e.g. HVAC systems).
Thermal radiation is the movement of heat energy from a warm object to a cooler item through space. A common example involves an occupant standing in front of a window and experiencing cold during the winter. In this instance, one’s body is radiating heat to the cooler window surface.
The importance of limiting heat transfer is not only to promote energy efficiency and occupant comfort, but also to reduce the potential for condensation within building assemblies. When moist air comes into contact with nonporous surfaces below the dewpoint, water can condense and create frost or water droplets (Figure 2).
Insulating materials are categorized by their resistance to heat flow, otherwise known as R-value. The higher the R-value, the greater the resistance to heat transfer. Another measure associated with heat transfer is the U-factor, typically associated with opaque assemblies or fenestration products. The smaller the U-factor, the better the product limits heat transfer.
Air transfer
The new codes include major changes regarding air transfer—not only because of a desire for increased energy efficiency, but also because air movement is often a large contributor to moisture migration.
Air has a natural tendency to move from high to low pressure (Figure 3). Sources of pressure differentials at a building envelope are diverse. For example:
- wind can create shifting positive and negative pressures on the enclosure;
- stack effect can exist with differences in atmospheric pressure between the top and bottom of the building; and
- fan pressure created by the HVAC system can also result in positive or negative pressure, depending on mechanical system design and operation.
Air carries moisture in the form of vapor, which can then encounter surfaces below the dewpoint and cause condensation. For air transfer to occur, there must be a path or a hole between two areas of differing pressure—either exterior to interior, or between varying interior conditions.
Air permeance is defined as the rate of airflow through a unit area of material under a given pressure difference. The performance of the air barrier materials is measured in L/(s•m2) (cfm/sf) of air leakage. Air barrier systems require complete continuity as any gap, hole, or crack allows for air transfer.
I had no idea that there were so many different types of air conditioners available. Based on what you said, a multi split unit might work best in my home since I have many smaller rooms. I’ll have to call a contractor and see what my best options are and how to install them. Thanks for the awesome advice and info!
Example of damage that can result from moisture accumulation. Images courtesy Raths, Raths, and Johnson : sbobetmessi
nsulating materials are categorized by their resistance to heat flow, otherwise known as R-value. The higher the R-value
I am definitely not very knowledgeable when it come to heaters and such. We are looking at getting a different heater in our home, and this article definitely helped me better understand the basics of heaters. I will be sure to share this information with my husband, especially the part about thermal control and the difference between different heater types.